Taking the measure of light (by Stephen Matthews)
Государственный комитет СССР по народному образованию
Т.И. Кузнецова, Г.В.Кирсанова
Методические указания по обучению чтению
технической литературы на английском языке по
специальности «Оптика»
Часть 4
(редакция 2008 года,
только для web-сайта факультета «Лингвистика»)
Издательство МГТУ
1991
MODULE 11 Q-SWITCHING Texts: A. Choosing the Best Alternative B. Acousto-Optic Q-Switching C. FO Strain Sensor to Watch for Quakes |
Terminology A:
1) Quality factor (Q) – коэффициент добротности, Q-switching – модуляция добротности, Q-switch – модулятор добротности, acousto-optic Q-switch – акустооптический модулятор добротности, electro-optic Q-switch – электрооптический модулятор добротности, rotating-element Q-switch – модулятор добротности с помощью вращающегося элемента;
2) shutter – затвор, intracavity shutter – внутрирезонаторный (внутриполостной) затвор;
3) energy storage capacity – количество запасенной энергии;
4) cell – ячейка, dye-cell Q-switch – модуляция добротности на ячейке на красителе, Kerr-cell Q-switching – модуляция добротности с помощью ячейки Kеppa, Pockels cell – ячейка Поккельса;
5) two-state saturation – двухуровневое насыщение, saturable absorber –насыщающийся поглотитель.
Vocabulary Practice
1. Combine the words from groups A and В to make terms:
A B
1) intracavity | 6) dye-cell | a) Q-switch | f) shutter |
2) quality | 7) power | b) time | g) density |
3) upper | 8) decay | c) holography | h) energy |
4) excess | 9) population | d) inversion | i) factor |
5) double-pulse | 10) range | e) finding | j) laser state |
|
|
2. Choose the correct word (or the form of the word) to complete the sentence that follows:
a) longer, no longer, any more, any longer
Stimulated emission __________ occurs, and lasing stops.
b) rise, raise, rose, risen, raised
If pumping continues, more atoms can be ________ to the upper laser level.
c) in use, in operation, in question
Operating parameters for each type of Q-switch depend on the material ________.
d) accessible, saturable, available, favorable
If the Q-switch is suddenly opened, that excess energy is _________ for immediate transmission.
TEXT 11A Q-SWITCHING. CHOOSING THE BEST ALTERNATIV
Short pulses of high peak power are useful in many applications of lasers – materials processing, rangefinding, and double-pulse holography are just a few examples. Some lasers generate such pulses as a matter of course. Others do not. Some of these other lasers can be induced to generate nanosecond pulses with peak powers of hundreds of megawatts by changing (i.e. switching) the quality factor, Q, of the laser cavity during pumping.
The key to Q-switching is energy storage capacity, a combination of optical gain and decay time. Generically, a Q-switch can be thought of as an intracavity shutter (Figure I). Under normal laser excitation and with the Q-switch open atoms are pushed into the upper laser state at some given rate, spontaneous emission begins to occur, optical feedback is provided by the two laser mirrors, and stimulated emission (laser action) ensues. Without getting deeply involved in rate-equation analysis, if the optical gain is larger than the optical losses and the various upper- and lower- level decay times are favorable, a steady-state lasing equilibrium can be achieved.
If the Q-switch is closed, the optical feedback is eliminated, stimulated emission no longer occurs, and lasing stops. But if pumping continues more atoms can be raised to the upper laser level than are found there under the condition of operational equilibrium just described. Stated simply, under the “closed-shutter” condition, excess energy is stored in the upper laser level. If the Q-switch is suddenly opened, that excess energy is available for immediate emission. The instantaneous rate of stimulated emission is quite high for the first photons reflected from the rear mirror, and a large laser pulse is emitted. This depletes the upper level and lasing stops until more energy can be placed in the upper laser level again.
|
|
The best laser candidates for energy storage are several types of solid-state lasers – Nd: YAG, Nd:glass, ruby, and so on. At the other extreme, ion lasers cannot be Q-switched: the population inversion in an ion laser decays so quickly that these lasers are unable tostore energy. Other types of gas lasers, such as carbon dioxide lasers, however, can be Q-switched.
Several types of Q-switches can be used. The three most common in terms of market penetration are acousto-optic, electro-optic and dye-cell Q-switches. The advantages and disadvantages of each are described in subsequent texts. Operating parameters for each type depend on the material in use.
At least two other types of Q-switches had been used in the past but they have little market presence today. Kerr-cell Q-switching, similar to electro-optic Q-switching but with a single polarizer, isn’t used very often anymore because of difficulties in dealing with materials like nitrobenzene and problems associated with component damage.
The other infrequently encountered type of Q-switching is the rotating-element Q-switch. If the rear mirror of the cavity were rotated in the plane of the paper (see Figure I) for a brief portion of each complete rotation, it would form a return path for optical feedback and lasing would occur. If this brief portion of rotation coincides with the period of peak energy storage in the laser medium, maximum peak power in the Q-switched pulse can be obtained. For the rest of each complete rotation, the cavity is nonresonant and lasing is inhibited (a retroreflective prism could also be used as the rotating element).
|
|
Figure 1. The generic Q-switch, which acts as an intracavity shutter. An infrequently encountered type of Q-switch is the rotating-element Q-switch, where the rear cavity mirror spins on an axis normal to the cavity axis.
With good optical coatings, rotating-element Q-switches can withstand higher power densities than can other types of Q-switches. However, difficulties in synchronization can make these devices difficult to work with, to the point where the user synchronizes the experiment to the Q-switch rather than vice versa. Another problem concerns angular velocity: top speeds of several hundred hertz lead to minimum pulse duration of the order of 30 nanoseconds. However, in the middle infrared, where materials capable of sustaining high-power operation are available for use as electro-optic or acousto-optic Q-switches, the rotating-element Q-switch still has a place. The recent interest in the erbium-doped YAG laser, which operates near 3 micrometers, may lead to a comeback for the rotating-element Q-switch.
4600 п. зн.
Review
1. Match the parts of sentences in column A with those in column В to produce true statements:
A B
1) Generally, a Q-switch can be thought of | a) the optical feedback is eliminated and lasing stops. |
2) If the Q-switch is open, | b) these lasers are unable to store energy. |
3) If the Q-switch is closed, | c) as an intracavity shutter. |
4) The best laser candidates for energy storage | d) spontaneous emission begins to occur. |
5) The population inversion in an ion laser decays so quickly that | e) is energy storage capacity, a combination of optical gain and decay time. |
6) With good optical coatings, rotating-element Q-switches | f) are several types of solid-state lasers: Nd:YAG, Nd:glass, ruby, etc. |
7) The key to Q-switching | g) can often withstand higher power densities than can other types of Q-switches. |
|
|
4. Pick out the words that do not belong to the following groups:
a) Nd:YAG, carbon dioxide, Nd:glass, ion, ruby;
b) dye-cell, rangefinding, electro-optic, acousto-optic, Kerr-cell, rotating-element;
c) rear cavity mirror, intracavityshutter, laser medium, energy storage capacity, flashlamp, output mirror, laser output;
d) Q-factor, shutter, polarizer, feedback, comeback, pulse, cavity, gain, emission.
5 . Study the following items of Text 11 А 's plan and reorder them according to the text:
1) Types of Q-switches.
2) The rotating-element Q-switch operation.
3) Basic concepts of Q-switching.
4) Advantages and disadvantages of the rotating-element Q-switch.
5) Processes that occur under different positions of a Q-switch.
6) Kerr-cell Q-switching.
7) Q-switching characteristics of different materials (types of lasers).
Discussion
6. Answer the questions to Text A:
1) List the applications of lasers with short pulses of high peak power. 2) What does changing the quality factor result in? 3) What is a Q-switch? 4) Say what happens under normal laser excitation and with the Q-switch open. What is the position of the Q-switch for lasing to stop? 5) What are the best laser candidates for energy storage? 6) Explain why ion laser cannot be Q-switched. 7) Enumerate the types of Q-switches described in the text. Which are the most common types? 8) Describe the operation of rotating-element Q-switches. What are their advantages? What makes these devices difficult to work with?
7. Use exercises 5 and 6 to sum up the contents of Text 11A.
Terminology B:
1) strain waves – волны упругости (упругие волны);
2) laserbeam deflector – лазерный дефлектор;
3) optical signal processor – устройство оптической обработки сигнала;
4) laser frequency shifter – устройство сдвига лазерной частоты;
5) mode-locking – синхронизация мод, mode-locker - синхронизатор мод, mode-locked laser system – лазерная система в режиме синхронизации мод;
6) bulk damage – здесь: разрушение (стойкость к разрушению) кристалла;
7) r.f. drive power - разночастотный источник сигнала.
Vocabulary Practice
1. Match the terms on the left with the Russian equivalents on the right:
1) mechanically induced strain waves | a) плотность на выходной мощности |
2) tunable optical filters | b) лазерный дефлектор |
3) single-pulse bulk-damage threshold intensity | c) длительность импульса лазера с акустооптической модуляцией добротности |
4) laser cavity configuration | d) перестраиваемые оптические фильтры |
5) output power density | e) плавленый кварц |
6) laserbeam deflector | f) волны упругости, наводимые механическим путем |
7) fused quartz | g) инверсная населенность вустойчивом состоянии |
8) steady-state population inversion | h) повреждение покрытия |
9) coating failure | i) схема лазерного резонатора |
10) acousto-optic Q-switched pulse duration | j) пороговая мощность разрушения при моноимпульсном излучении |
2. Match the verbs on the left with the definitions on the right :
to mature | to think about something carefully; to ponder; to study; to observe or examine; |
to inhibit | to say that something will happen, before it happens; to foretell; |
to predict | to become ripe; to reach completion; |
to provide | to prevent something from growing or developing well; to restrain; to hinder; |
to consider | to bring about or ensure; to arrange for smth or prepare beforehand; to supply smth. |
TEXT 11B ACOUSTO-OPTIC Q-SWITCHES
The term “acousto-optic” describes the interaction of optical waves with mechanically induced strain waves, which vary the index of refraction in an optical interaction medium. This type of interaction was first predicted by Brillouin in 1922. But it was not until after the development of the laser in the early 1960s that acousto-optic devices began maturing into the products of today. The acousto-optic interaction has led to the development of many useful devices: laser modulators, laserbeam deflectors, optical signal processors, tunable optical filters, laser frequency shifters, modelockers, and Q-switches.
Of particular interest is the acousto-optic Q-switch; consider the laser cavity configuration shown in Figure 2. If the laser medium is continually pumped without any optical feedback mechanism, a steady-state population inversion will be established in the laser transition level. An excess population of this level can be obtained if the radiative lifetime is long and the pumping intensity is high. The addition of the two end mirrors will form a cavity and provide the required feedback for lasing. The properties of the laser cavity are characterized by the quality factor, Q. A high value of Q means that energy is stored in the cavity with only very small losses. A low value of Q, conversely, indicates the presence of large energy losses is the cavity, and the lasing will be inhibited.
Figure 2. Simplified schematic of an acousto-optic Q-switched laser cavity
For the acousto-optic Q-switched laser configuration shown in Figure 2, the Q factor is given by the expression Q = 2d ω/(I – R + Rŋ)c , where R is the reflectivity of the output mirror, d is the distance between the mirrors, ω is the angular frequency of the light, с is the velocity of light, and ŋ is the fraction of light diffracted out of zero order when the Q-switch is on.
With rf drive power applied to the Q-switch, ŋis high andthe value of Q is held low, preventing lasing. In this state the laser medium has time to build up an excessive population in the laser transition level. After pumping the laser rod to the maximum population the rf drive is switched off and all the light is allowed to pass between the mirrors. This allows the laser pulse intensity to build up rapidly to the maximum and then decay as the excited states are depleted. This cycle is repeated at the desired laser pulse rate as limited by the optical and electrical time constants.
Acousto-optic Q-switches are commonly used in continuous-wave, modelocked, and pulsed laser systems to generate intense bursts of laser light. Q-switching usually reduces the energy of the pulses but at the same time the pulse length is shortened so much that the peak power is increased considerably. Acousto-optic Q-switched pulse durations typically range from 40 to 200 nanoseconds, depending on the laser design, Acousto-opticQ-switched ruby and Nd:YAG lasers produce output power densities of 107 to 108 watts per square centimeter; measurements as high as 4 x I09 W/сm² have been reported in simultaneously Q-switched and modelocked systems. By using a variety of optical interaction materials Q-switches have been designed to work in laser systems operating at wavelengths ranging from 300 nanometers to 11 micrometers.
Fused quartz is the most commonly used acousto-optic Q-switch medium for operation between 300nmand 2μm. The single-pulse bulk-damage threshold intensities at wavelengths of 1064 and 532 nm are 110 and 90 GW/cm², respectively. Multi-pulse balk damage occurs at about 80 percent of threshold at 1064 nm and 60 percent of threshold at 532 nm, as reported by Merkle et al.[1] of the University of Southern California. Interestingly, quartz optical elements typically suffer coating failure before bulk damage occurs. For applications requiring low optical power more efficient materials, such as lead molybdate (PbMoO4) and flint glass[2] are used in some devices operating between 600 and 1200 nm. However, these materials find limited use because of their high internal optical absorption.
Constant improvements in acousto-optic Q-switch design and fabrication techniques are leading to more efficient, reliable and more stable systems. However, the final Q-switch choice will be dictated by the user’s system requirements and economic tradeoffs.
Review
3. Match the parts of sentences in column A with those in column В to produce true statements:
1) The term “acousto-optic” describes | a) the presence of large energy losses in the cavity. |
2) It was not until after the development of the laser in the early 1960s | b) energy is stored in the cavity with only very small losses. |
3) An excess population of the laser transition level can be obtained | c) the interaction of optical waves with mechanically induced strain waves. |
4) A high value of Q means that | d) because of their high internal optical absorption. |
5) A low value of Q indicates | e) range from 40 to 200 nanoseconds, depending on the laser design. |
6) Acousto-optic Q-switched pulse durations typically | f) that acousto-optic devices began maturing into the products of today. |
7) These materials find limited use | g) if the radiative lifetime is long and the pumping intensity is high. |
4. Pick out the words that do not belong to the following groups:
a) continually, conversely, rapidly, commonly, reflectivity, considerably, typically, simultaneously, respectively, interestingly;
b) shorten, reduce, limit, deplete, build up, decay;
c) transition level, interaction medium, drive power, pulse rate, time constant, index of refraction, bulk damage, power density;
d) inhibit, prevent, stop, hinder, provide.
5. Study the items of Text 11 В ’s plan and reorder them according to the text. Pick out the items that have nothing to do with this text:
1) Lasers using acousto-optic Q-switches.
2) Operation difficulties of dye-cell Q-switching.
3) The expression for the Q factor.
4) Prospects of developing more stable systems.
5) Acousto-optic Q-switch operation. Simplified version.
6) Outstanding electro-optic crystals.
7) Acousto-optic interaction. Background.
8) The most commonly used acousto-optic Q-switch media.
6. Write an abstract: of Text 11B.
Discussion
7. Answer the questions to Text 11B:
1) Who was the first to predict acousto-optic interaction? What does the term mean? What devices are based on this interaction? 2) Describe the operation of the acousto-optic Q-switch. Use Figure 2 and the expression for Q factor. 3) What types of laser systems commonly use acousto-optic Q-switches? 4) What is the most commonly used acousto-optic medium? What applications require the use of other materials? Why?
8. Compare the configuration and operation of the rotating element Q-switch (Text 11A, Figure 1) with those of the acousto-optic Q-switch (Text 11B, Figure 2).
9. Render the text given below in English.
Коэффициент добротности Qопределяется потерями лазерного резонатора; чем меньше потери, тем выше значение q. Рассмотрим лазерный резонатор, в котором перед одним из зеркал помещен затвор. При условии постоянной накачки активной среды и при закрытом затворе инверсная населенность активной среды в резонаторе продолжаетувеличиваться и достигает максимального значения. Если затвор закрыть, то величина инверсной населенности будет соответствовать начальному, гораздо выше порогового, значению; энергия, запасенная резонатором, будет выделяться в виде короткого импульса света с высоким максимальным значением интенсивности. Поскольку при открывании затвора значение Q возрастает от очень малой величины до очень большой, эта методика получения короткого интенсивного импульса света получила название мгновенной модуляции добротности.
10. Read the following text and answer the questions:
1) What is the sensitivity of the system described? 2) What types of laser are used in the system? Why? 3) List possible applications of the fiber strain sensor.
TEXT 11C FO STRAIN SENSOR TO WATCH FOR QUAKES
A fiberoptic strain sensor being developed at the Los Alamos National Laboratory will watch for small strain in the earth’s crust, which may precede earthquakes. The system сan detect strains as small as 10-10, which can develop over a period of years.
To achieve that sensitivity Los Alamos geophysicist Fred Homuth turned to an interferometric sensor based on two parallel singlemode fibers installed without cabling in a hole in the ground. One is isolated from the influence of the surrounding rock; the second is cemented to the rock so it experiences strains in the surrounding rock. Strain-induced alterations (изменения) in the effective path length of the second fiber are detected by comparing its output with that of the reference fiber interferometrically and continually monitoring the output with a computer.
The sensing system will use about 200 meters of a special singlemode fiber with wavelength of 1095 nm. That special fiber is needed because Homuth is using helium-neon laser sources emitting at 1152 nm. Those lasers were selected for their high stability and the low fiber losses at that wavelength.
Homuth noted that the fiber strain sensor could find other applications including detecting underground nuclear explosions, monitoring ground stability near waste storage and nuclear reactor sites, and monitoring stability of structures such as pipelines built on permafrost.
1500 п.зн.
SUPPLEMENTARY READING TASKS
Q-SWITCHING: THE ELECTRO-OPTIC ALTERNATIVE
Electro-optic Q-switching are used with virtually all high-power pulsed solid-state lasers and some infrared gas lasers that operate in the Q-switched or cavity-dumped modes. First applied in the early 1960s, electro-optic Q-switches have evolved into a variety of configurationsand several crystal materials.
Typically, electro-optic Q-switching can be attained in a laser cavity having sufficient gain to sustain oscillation with the Q-switch in the optical path when the switch is in the “open” condition. Conversely, since a minute amount of optical leakage through the Q-switch almost always occurs when the switch is in the "off" condition, the "off"-state gain must be low enough to prevent the buildup of oscillations. Leakage, with subsequent pre-lasing, can be a problem with heavily pumped Nd:YAG lasers. The most common arrangement of components for Q-switching is the quarterwave configuration, wherein the voltage applied to the electro-optic crystal is just enough to induce quarterwave retardation in polarization at the laser wavelength.
The sequence of events is straightforward. First, quarter-wave voltage is applied to the Q-switch. After voltage is established, the flashlamp is fired and the laser rod is pumped to store energy. While quarterwave voltage is applied tothe Q-switch, the roundtrip gain in the cavity is low; the cavity isin a lossy state. That is, the cavity quality factor, Q, is low and no oscillation is taking place. Next, while energy builds in the laser rod, the rod begins to emit spontaneously. This emission passes through the polarizer and becomes linearly polarized. Then, the beam passes through the Q-switch and be-comes circularly polarized (quarterwave retardation corresponds to circular polarization). After being reflected at the 100-per-cent reflective mirror, the beam again passes through the Q-switch and undergoes another quartarwave retardation. The resulting linearly polarized beam is polarized at 90° to the original polarization. The new direction of polarization is blocked and the beam is deflected out of the laser cavity through a side escape window on the polarizer. This prevents the optical feedback necessary со build up oscillations in the cavity. Laser emission is thereby inhibited.
While the cavity is in a lossy state, the flashlamp continues to рumр the laser rod until a level described as maximum population inversion is reached. At this level, additional flash-loop pumping cannst increase the amount of energy stored in the rod and only contributes to raising the temperature of the rod. Just after the population inversion is maximized and before the flashlamp is turned off, the voltage across the Q-switch is switched to zero (in about 5 to 10 ns). Now, the beam passes through the crystal without retardation, oscillations build up in the cavity, and a Q-switched pulse in generated.
Q-SWITCHING: THE DYE-СELL ALTERNATIVE
Of all the Q-switching techniques, by far the least expensive to implement is the dye-cell Q-switch. Unlike the others, which require complex electronic drivers and synchronization circuitry, the dye-cell Q-switch only requires the mixture of an appropriate organic dye and solvent and an intracavity cell to hold that mixture.
With most materials, optical transparency does not change appreciably with optical intensity until very high intensities are reached, when bulk damage can occur. Certain organic dyes, properly called saturable absorbers, are the opposite. For saturable absorbers, optical transparency is very low at low optical intensities. But as the optical intensity increases to modest levels (well below the very high intensity levels typical of damage thresholds in most materials), the absorption coefficient of the saturable absorber begins to drop sharply, and the material becomes increasingly transparent. This effect is often celled bleaching.
If such a material is placed inside a laser cavity, it will act as a closed shutter during the early buildup of laser action, blocking the optical feedback path by shielding one of the mirrors. But as the intensity of spontaneous emission along the optical axis increases (in step with reaching high levels of energy storage within the laser medium), the saturable absorber will quickly become transparent. This opens the optical feedback path, laser action quickly ensues, and the excess energy stored in the laser medium is quickly depleted, as with other types of Q-switches. As a practical matter, this limits dye Q-switching mostly to pulsed solid-state lasers, because continuous wave solid-state lasers typically do not produce enough intensity to bleach a saturable absorber. In keeping with the analogy of a Q-switch as an intracavity shutter, the dye-cell Q-switch would be a self -actuated shutter, unlike the electro-optic or acousto-optic versions which need external electronic control.
DYE-CELL Q-SWITCHES: OPERATIONAL DIFFICULTIES
If the foregoing (see text 2) were all there is to dye-cell Q-switching, other forms of Q-switching would not be used except for special cases. But the properties of the relatively few dyes useful for Q-switching solid-state lasers contribute to operational difficulties.
First of all, candidate dyes must have an absorption peak coincident, or nearly so, with the laser emission wavelength. As a practical matter, dyes used to Q-switch ruby or alexandrite lasers won't Q-switch Md:YAG or Nd:glass lasers. Also, while a fair number of organic dyes are available for Q-switching ruby or alexandrite lasers, only a handful of dyes with absorption peaks near 106O nanometers have been synthesized for use with Nd:YAG lasers.
Second, most dyes useful for Q-switching solid-state lasers are susceptible to breakdown from ultraviolet light. Care in storing the dyes is essential, as is the inclusion of an ultraviolet filter in the cavity to shield the dye from the output of the flashlamp. Often, this filter is a coating incorporated in the dye cell.
Third, the simplicity inherent in not having electronic drivers for dye Q-switches comes at a price: timing jitter. Because the statistics of laser buildup vary from pulse to pulse, the exact number of cavity round-trips before maximum bleaching occurs will also vary. This has the effect of causing jitter in the timing of the output pulse relative to the start of the pump pulse. Any required synchronization must be done relative to the output pulse, using optical delay paths.
Fourth, the most difficult to deal with, the properties of the laser output from a dye-Q-switched laser depend strongly on the relaxation properties of the specific dye used. If the relaxation time is of the order of a cavity roundtrip time, typically a few nanoseconds, Q-switching is simple. But if the dye relaxes more quickly, say tens of picoseconds or less, it will tend to modelock rather than Q-switch the laser output. The key to encouraging modelocking behavior with fast-relaxation dyes is to design the laser cavity with all the intracavity components at Brewster’s angle; the key to suppressing modelocking behavior (in favor of Q-switching behavior) with such dyes is to design the laser cavity with all the intracavity components at right angles to the optical axis. In other words, polarization plays a key role in the Q-switching/modelocking of fast-relaxation dyes. Several dyes capable of Q-switching Nd:YAG lasers fall into this category. Other relax slowly enough to permit the switching of Nd:YAG lasers in the nanosecond regime.
While the discussion has stressed the use of dyes аs saturable absorbers for solid-state lasers, the saturable absorption effect is not limited to those materials and wavelengths. Carbon dioxide lasers emitting at the 10.6-micrometer band can be Q-switched by certain gases exhibiting saturable absorption. Perhaps the most commonly used gas for this task is sulfur hexa-fluoride. Q-switched pulse durations typically are about 1 to 2 ns.
MODULE 12 MODELOCKING Texts: A. Compact Picosecond Nd: glass Mode-Locked Laser with Variable Cavity Length B. Pulse Shortening by a Nonlinear Mirror-Mode Locker C. Transverse and Longitudinal Mode Selection |
Terminology A:
1) mode - locked laser – лазер в режиме синхронизации мод;
2) pulse-to-pulse spacing – расстояние между импульсами;
3) remote sensing – дистанционное зондирование;
4) gain depletion – затухание усиления;
5) shot-to-shot optical excitation – эффективность оптической накачки;
6) extender mirror – зеркало для увеличения оптического пути.
Vocabulary Practice
1. Combine the words from groups A and В to make terms:
A B
1) brewster | 6) remote | a) stability | f) amplifier |
2) steady-state | 7) absorber | b) sensing | g) angle |
3) environmental | 8) pulse-to-pulse | c) method | h) effects |
4) shot-to-shot | 9) matrix | d) train | i) passes |
5) successive | 10) pulse | e) separation | j) cell |
2. Fill in the blank spaces with the word-combinations that follow:
in essence – в сущности, по существу; in comparison with – по сравнению; in general – в общем, вообще; in order to - (для того) чтобы; in addition (to) – кроме того.
1) __________ produce long pulse train it is required to obtain an ultralong cavity.
2) ___________the above mentioned methods there exist many other techniques for mode selection.
3) The effective cavity length of the compact mode-locked laser _________ the conventional one is from 5 to 21 m.
4) In some cases the oscillator can act as an amplifier to the optical pulse which__________ overcomes additional losses in the cavity.
5) Such oscillator could ___________be referred to as a steady-state amplifier.
TEXT 12A COMPACT PICOSECOND Nd: GLASS MODE-LOCKED
LASER WITH VARIABLE CAVITY LENGTH
I. Introduction
For over 20 years solid-state mode-locked lasers have been the workhorse for investigating picosecond and nonlinear optical phenomena. A typical solid-state mode-locked laser such as Nd:glass produces a pulse train lasting for 100ns of high peak power (2-GW) pulses of 8 ps duration. In a conventional mode-locked laser the cavity length ranges from I to 2 m due to available limited space and mechanica1 stability. The pulse separation in such a conventional mode-locked laser is between 5 and I0 ns. To determine the time dependence of phenomena occurring in materials without interference from other exciting pulses in the pulse train a single pulse is usually extracted from the train of pulses produced by the laser using an electro-optic device. For certain situations it is desirable to have an ultralong pulse-to-pulse spacing for applications such as ranging and remote sensing to mention a few. Long pulse-to-pulse separation is important to reduce the effect of gain depletion and shot-to-shot optical excitation of samples. To produce such a pulse train requires an ultralong cavity. The folding of a cavity reduces the overall length.
This text reports on a new compact mode-locked laser design with an effective cavity length from 5 to 2I m. The performance and characteristics of the new laser such as parameters of energy, number of pulses, length of train and stability of the cavity are presented.
II. Experimental Setup
A diagram of the compact design is shown in Fig.3 Brewster angle cut neodymium doped silicate rod and saturable absorber cell is located between several mirrors making up the cavity. The overall geometrical length of the laser is less than 1.7 by 0.3 m wide. The cavity contains a rear mirror (M4) with a radius of curvature of I.5 m, three spherical optical path extender mirrors (M1 – M3), each with a radius of curvature of 1 m, and an output mirror (M0). The rear mirror and optical path extender mirrors were all coated for 100% reflectivity at 1.06 m. The output mirror (M0) was coated for 65% reflectivity. The mode-locking dye cell was filled with Kodak 9860 dye dissolved in 1.2-dichloroethane with transmission set at 70%.
The pulse train length and spacing were measured using an ultrafast Hamamatsu (CI083) PIN diode coupled to a Tektronix 519 oscilloscope. The pulse duration was measured with aHamamatsu C979X streak camera coupled to a video analyzer. The pulse train energy for each cavity length was measured using a Laser Precision Energy meter (RK-3230). Shot-to-shot stability of the laser appeared to be excellent for all cavity spacings.
The three optical path extender mirrors (M1-M3) were placed between the back mirror (M4) and the dye cell. This provided a means of varying the optical path length of the cavity without changing its geometrical length. The distance between the mirrors was as follows: M0 – M1 was set at 1.7 m, M1 – M2 and M2 – M3 were set at 1 m each, and M3 – M4 was set at 1.65 m.
III. Description of Cavity Design
To describe how the beam profile changes within the cavity a сingle pass through the path extender section is considered for simplicity. A collimated beam originating at the laser rod reflects off mirror M1 and focuses to the midpoint (at 0.5 m) between mirrors M1 and M2. Mirror M2then recollimates the beam, then travels and reflects off mirror M3. Mirror M3 then focuses the beam to apoint 0.5 m away which is located at the radius of curvature of mirror M4. The beam in turn reflects off M4 and refocuses at the same point located 1.5 m from mirror M4. The beam then retraces its path back through the cavity.
The transverse structure appears to be inform due to the inherent refocusing and recollimating properties of the M1, M2, M3, and M4 mirror arrangement and the location of the apertures.
By adjusting mirror M3 the cavity length can be changed in 4-m steps corresponding to 13 ns/step. The travel time for apulse through the beam path extender section for N reflections off the central mirror M2 is Т = (2N + 2) L/c, where L is the distance between mirrors M1 – M2 and M2 –M3 .The path lengths were measured from the pulse trains to be 5.25, 9.25, 13.25, 17.25 and 21.25 m.
IV. Experimental Results
Laser thresholds, pulse-to-pulse time spacing and pulse energies with respect to the number of passes through the path extender mirrors and the effective optical path length are summarized in Table 1. The duration of a pulse early in the train is 10.5 ps.
For various passes through the path extender section of the cavity the threshold energy increased slightly from 3.2 to 4.7 kVwhile the output energy remained fairly constant at 550mJ with the exception of nine passes (N = 9). For N = 9 passes one of the reflections ran off the edge of the central mirror (M2) resulting in a decrease in energy at the output.
The pulse train length was found to be extremely stable and long, of the order of 5 μs.This was attributed to the high stability of the cavity and long pulse separation, which allows the lasing medium ample time to recover and repopulate between successive passes of the optical pulse. In this case, the oscillator appears as an amplifier to the optical pulse, which in turn overcomes additional losses in the cavity due to multiple reflections off each mirror. This may be viewed in essence as a steady-state amplifier.
V. Applications
Pulse-to-pulse spacings can easily be selected in steps of I3.2 ns with the realignment of only one mirror (M3), no other mirror is required to be realigned. Fоr longer (or shorter) pulse separations this design can be scaled. With this cavity design we achieved a long optical path length in a short geometrical length (1.7 m) thereby minimizing environmental and mechanical effects providing a very stable configuration. The physical size, stability, and elimination of devices external to the cavity are clear advantages over the standard two-mirror cavity designs. Applications may include dynamic remote sensing and ranging of objects through the modulation of the pulse train.
VI. Summary
In summary, the overall size of the new laser design is 1.7 m long by 0.3 m wide. The pulse-to-pulse spacing is variable from ≈ 35 to I40 ns. The train length was of the order of 5 with ≈100 pulses. The pulse duration of early pulses was measured to be 10.5 ps. The stability diagram for the cavity wascalculated using the matrix method.
6700 п. зн.
Review
3. Match the parts of sentences in column A with those in column B to produce true statements:
1) The cavity length of a conventional mode-locked laser | a) a rear mirror, three optical path extender mirrors and output mirror. |
2) The transverse structure appears to be uniform due to | b) with a Hamamatsu camera coupled to a video analyzer. |
3) The cavity contains | c) using the matrix method. |
4) The pulse duration was measured | d) to recover and repopulate. |
5) The stability zone for the cavity is calculated | e) dynamic remote sensing and ranging of objects. |
6) The high stability of the cavity and long pulse separation allows the lasing time | f) the inherent refocusing and recollimating properties of the mirrors. |
7) As for applications, they may include | g) ranges from I to 2m. |
4. Pick out the words that do belong the following groups:
a) refocus, recollimate, retrace, remain, repopulate, realign, reconvert;
b) pulses, trains, results, samples, ranges, radians;
c) integration, cooperation, combination, unity, separation.
5. Study the following sentences and reorder them according to the text to obtain a logical abstract
1) The overall size of the laser is 1.7 m.
2) The stability zone for the cavity is calculated using the matrix method.
3) The design, performance and characteristics of a compact mode-locked laser with effective cavity length of 5 to 21 m is described.
4) The pulse duration was measured to be 10 ps for the early pulses in the train using a streak camera system.
5) Pulse-to-pulse spacing of ≈ 35 =140 ns is obtained.
6) The laser parameters of energy, number of pulses, length of train and stability of the cavity are described.
Discussion
1) Compare all the characteristics of a conventional mode-locked laser with those of the reported one. What are the advantages of the latter?
2) Study carefully the diagram of the compact design in Fig.3. Is it different from the design of a conventional solid state mode-locked laser? What are the differences?
3) Applications mау include dynamic remote sensing and ranging of objects to mention a few. Do you know any other applications? Could they bе possible in case of a typical mode-locked laser?
Terminology B:
1) intensity - dependent light transmission – управляемое светоиспускание;
2) walk-off effect – эффект блуждания;
3) double - pass generation – двупроходимая генерация.
Vocabulary Practice
1. Find the equivalents of the word - combinations in Text 12 B:
разделение амплитуд, произвольное отношение, быстрое реагирование, идеальное фазовое соответствие, эффективность мгновенных преобразований, более отчетливо выражать, грубые (приближенные) оценки, круговые пробежки, за пределами темы данной статьи, заслуживает упоминания, технология пассивной синхронизации мод.
2. Translate the sentences to obtain the abstract of Text 12 B:
Прибор, состоящий из нелинейного кристалла и дихроичного (глухого) зеркала, обладает особенностью управляемого отражения и может использоваться как пассивный синхронизатор мод. Было рассчитано укорачивание импульса для нормализованного Гауссова импульса, обусловленное отражением с помощью нелинейного зеркала. Сделано сравнение с показателями насыщающегося поглотителя, используемого для синхронизации мод.
TEXT 12В PULSE SHORTENING BY A NON-LINEAR MIRROR MODE LOCKER
Saturable absorbers exhibit intensity-dependent light transmission. In mode-locked lasers this feature is used to perform amplitude discrimination and pulse shortening. Pulse shortening has been analyzed in the case of a fast absorber, in the intermediate case, and has been calculated for an arbitrary ratio of the pulse duration to the absorber relaxation time.
Recently a novel nonlinear optical device utilizing second harmonic generation was proposed. It may exhibit intensity-dependent reflection or transmission with a fast time response in the subpicosecond range. Successful mode locking of a Nd:YAG laser using this device was demonstrated. Since one of the important characteristics of a mode locker is pulse shortening in a single transit, in this paper we calculate pulse shortening due to reflection by the nonlinear mirror and make a comparison to the performance of a saturable absorber used in a mode-locked laser.
The nonlinear mirror is a combination of a nonlinear crystal generating second harmonic and a dichroic mirror placed behind it. The incident light generates second harmonic in the crystal and the dichroic mirror reflects totally the second harmonic and partially the fundamental radiation back into the nonlinear medium. Same means are provided to adjust the phase differences φ = 2 φ1 - φ2 (φ1, φ2 are the phases of the fundamental and second harmonic waves) to be -π/2 + 2mπ. After reflection by the dichroic mirror in the second pass through the crystal a reconversion of the second harmonic into fundamental takes place. The reflection of this device in these conditions increases by increasing the light intensity.
If the phase difference φ = π/2 + 2mπ, in the second pass further conversion into second harmonic is provided. Then, the action of the device is equivalent to a double-pass second harmonic generation with some modification of the wave intensities after the first pass. In this case the reflected intensity decreases by increasing the input one.
The analysis shows that both higher conversion efficiency and lower reflectivity of the dichroic mirror at the fundamental wavelength enhance the nonlinearity in light reflection. An expression for the nonlinear reflection coefficient at the fundamental wavelength Rnl as a function of the conversion efficiency ŋ and the dichroic mirror reflectivities at the fundamental, RI, and the second harmonic, R2, has been derived for the case of interest, i.e., when. φ = -π/2 + 2mπ. If the dichroic mirror is totally reflecting at the second harmonic, the nonlinear reflection is given by
The reflection coefficient RI may be regarded as a small-signal reflection analogous to the small-signal transmission of a saturable absorber, if we consider a light pulse reflected by the nonlinear mirror, the high-intensity parts of the pulse will be more efficiently reflected than the low-intensity parts. Hence, one expects pulse shortening, similar to that experienced by a light pulse passing through a saturable absorber. In accordance with the above brief discussion on the effect of the phase difference it is evident that the pulse shortening maximizes for φ = -π/2 + 2mπ. Deviation from this will reduce the pulse shortening and approaching φ = π/2 + 2mπ pulse broadening will result.
In what follows, we calculate the pulse shortening of a normalized Gaussian pulse with FWHM = 1 for ξ = -π/2 + 2mπ (2). A phase difference change within +/- (п/6) reduces the modelocking probability to ≈50%.
We confine our treatment to plane waves and perfect phase matching. The walk-off effect is compensated, since after reflection by the dichroic mirror the fundamental and second harmonic beams travel exactly the same optical paths. Thus, the only influence of the walk-off effect is reduction of the conversion efficiency. In addition, we assume pulse duration for which the crystal length is smaller than
are the group velocities for the fundamental and the second harmonic. This assures that the group velocity mismatch does not broaden the second harmonic pulse. For reasonably high conversion efficiencies the pulse durations in these conditions are in the 0.2-5-ps range, depending on the type of crystal and the wavelength region. Higher-order effects like dispersion spreading are neglected.
First we analyze the pulse shortening as a function, of the peak conversion efficiency. Thus, the incident Gaussian pulse with FWHM = 1 has the form
.
We use the following рrocеdure; the instantaneous conversion efficiency ŋ (t) is determined using the relation
where ŋ is the peak conversion efficiency at t = 0 for a pulse of unity peak intensity. Next, the instantaneous nonlinear reflection coefficient R (t) is calculated, substituting Eq. (4) into Eqs. (I) and (2). The reflected pulse form Ir(t) (at the exit of the nonlinear mirror, i.e., at the left-hand side of the crystal NLС) is given bу Ir(t) = I(t)Rnl(t) (5).
Lastly, we calculate the time at which the reflected intensity drops to half of its maximum value. The full wilth at half-maximum of the reflected pulse is a function of the peak conversion efficiency of the incident pulse for various values of the dichroic mirror reflectivity HI at the fundamental wavelength. As one can expect, the pulse shortening is more pronounced for high conversion efficiency. However, it peaks conversion efficiency in the range of 0.6-0.8 and then rapidly approaches the incident pulse duration. This is to be expected, since for a very high conversion the second harmonic pulse follows closely the pulse form of the fundamental (of course not in the wings) due to saturation.
By inspection of the expression for the reflected pulse form Eq. (5) it can be seen that the pulse shape is modified by the nonlinear reflection coefficient, which is a rather complex function. Thus, the reflected pulse shape does not follow the input one.
It is also evident that the pulse shortening is higher for lower reflection coefficient RI. This is in accordance with the features of the nonlinear mirror mentioned above. Here we remind the reader that the pulse shortening and the amplitude discrimination starts at conversion efficiency of the order of several percent as the mode-locking experiment has revealed. A reasonable question is why is the pulse shortening not close to аs one can expect for low conversion efficiency. It is well known that for a Gaussian pulse after the first pass through the nonlinear crystal the second harmonic pulse is shortened with respect to the fundamental by this factor. The reason is that the fundamental pulse will broaden because of the high intensity parts. In the second pass through the crystal the broadened fundamental pulse is amplified in the field of the second harmonic pulse, thus the effect of the pulse shortening in the second harmonic generation is somewhat reduced.
Finally, we illustrate pulse shortening as a function of the incident peak pulse intensity. Conversion efficiency of 0.5 is assumed for a pulse having unity peak intensity.
This part of the paper is devoted to a comparison of pulse shortening by the nonlinear mirror to that оf a saturable absorber when used аs a mode looker. The advantages of the nonlinear mirror such as simplicity, reliability, wide spectral ranges of operation, simultaneous generation of ultrashort light pulses at the second harmonic, and fast time response have been discussed. Because of the fast time response one might expect better performance of the nonlinear mirror in comparison with alow absorbers, at least for solid-state lasers. Therefore we compare pulse shortening to that of a fast absorber. When a single-pass extracavity pulse shortening is required, saturable absorbers of very low small-signal transmission of the order of 10-7 are superior and may provide an optimized pulse reduction by a factor of 3. However, in mode-locked solid-state lasers, the small-signal transmission ranges from 0.5 to 0.8. The optical arrangement with an absorber corresponding to the nonlinear mirror is a dye cell with a totally reflecting mirror behind it. Since the light passes twice through the cell, the low intensity transmission is 0.25-0.64. Thus, the corresponding relative pulse shortening [defined as (T - Tp )/Tp] may reach values in the range from -0.16 to -0.06. Here wenote that these are the maximum values attainable at an optimized intensity level only.
Considering a nonlinear mirror, intracavity conversion efficiencies of 0.5 can be realized with highly efficient nonlinear crystals such as KTP and BBO. Fоr RI ≈ 0.25-0.64 (small-signal reflection) the relative pulse shortening is in the range from -0.11 to -0.05, i.e., somewhat smaller but very close to that of the absorber. However, in a mode-locked laser the absorber pulse shortening maximizes at a certain intensity level, while the nonlinear mirror one increases monotonically for all realistic values of intensities and conversion efficiencies.
To reveal the ultimate capabilities of the nonlinear mirror with respect to pulse shortening one has to perform an analysis assuming nonstationary second harmonic generation and reconversion. However, some crude estimates are possible. For the experiment where 5.5-mm long KTP crystal was used, effective pulse shortening may be expected for pulses as short as 4 ps. especially favorable is the situation for mode locking an Nd: glass laser using a KDP crystal. In this case for a 2-cm long crystal pulses as short as 200 fs may still undergo pulse shortening. In both examples one can expect generation of ultrashort pulses with a duration determined by the spectral gain profile (if there are no other limitations, such as self-phase modulation or limited number of round trips). A comprehensive analysis of laser dynamics, including the effect of the nonlinear mirror and laser active medium, is out of the scope of this paper.
It is worth mentioning that the change of phase difference does not limit the bandwidth of the device, but only changes the direction of the interaction between the lightwaves in the second pass, i.e., reconversion back into fundamental or further conversion into second harmonic. Also, since the group velocity dispersion in air is several orders of magnitude less than in nonlinear crystals, the crystal mirror separation does not impose any practical limitations on the performance of the nonlinear mirror mode locker with respect to the bandwidth.
10700 п. зн.
Rewiew
1. Read text 12 В attentively. Divide it into logical sections (see Text 12A).
2. Think of a good title for each section.
3. Mark phrases which best serve as the main idea of each section. Give reasoning for your choice.
4. Combine the topic sentences and write a summary of the text.
5. Speak on the problems presented in Text 12B:
1) Analysis of pulse shortening due to reflections by a nonlinear mirror.
2) Comparison of the performance of the nonlinear to that of a saturable absorber mode locker.
3) Advantages of the new mode-locking device.
6. Look through Text 12C and answer these questions:
1) What does the mode selection depend on? 2) What type of the modes is the most preferable to work with and why? 3) Why is it necessary to make laser oscillate in the lowest order mode? 4) What influences the laser output directionality? 5) How does the volume of the active material depend on the cavity length?
TEXT 12 С TRANSVERSE AND LONGITUDINAL MODE SELECTION
Since optical resonators have dimensions which are large in comparison to the wavelength of light, there will, in general, be a large number of modes which fall within the atomic linewidth and which can oscillate in a laser. Thus the output say consist of a number of longitudinal modes each oscillating at a different frequency. Also, since the various transverse modes have different phase and amplitude distributions across the output plane, the resulting laser output may not be highly directional. In fact the power per frequency and solid angle interval is maximum when the laser oscillates in - single mode which is the fundamental mode. In order to obtain highly directional and spectrally pure laser output, various techniques have been developed both for transverse andlongitudinal mode selection in lasers. In this section we will briefly discuss some of the important mode selection techniques.
Transverse Mode Selection
As we showed above, the lowest-order transverse mode has a Gaussian amplitude distribution across a transverse plane. It is this mode that one usually prefers to work with as it has no abrupt phase changes across the wave front(as the higher-order transverse modes have) and has a monotonically decreasing amplitude away from the axis. This leads to the fact that it can be focused to regions of the order of the wavelength of light, producing enormous intensities. The Gaussian mode has the narrowest distribution and hence if an aperture is introduced in the cavity which increases the loss of the next higher order transverse mode to a value where the loss exceeds the gain, the laser would oscillate only in the lowest-order mode. Observe that this introduces additional losses even for the lowest-order mode. Specific higher-order transverse modes can also be selected at a time by choosing complex apertures which introduce high loss for all modes except the required modes or by profiling the reflectivity of one of the mirrors to suit the required mode. Thus, a wire placed normal to the axis and passing through the resonator axis would select the second-order TEOI mode.
Longitudinal Mode Selection
Even when a laser is oscillating in a single transverse mode, it could be oscillating in different longitudinal modes separated by a frequency spacing of c/2d. One possible method to obtain single-mode oscillation is to decrease the length of the cavity d to such a value that there is only one longitudinal mode within the gain profile which has its gain higher than the losses. Such a method can be used with gas lasers which have relatively small linewidths. It must be noted that as the length of the laser cavity is decreased, the volume of the active material also decreases and hence the output power would also decrease.
In solid state lasers the bandwidth is large and the above technique becomes, impractical. Hence various other techniques have been envolved for longitudinal mode selection.
3000 п. зн.
7. Translate the text below in writing using a dictionary:
TEXT 12D HYBRID SYSTEMS
Hybrid techniques generate shorter pulses than the corresponding synchronously pumped systems. The hybrid systems incorporate a saturable absorber folded section in the standard synchronously pumped cavity arrangement. Critical submicrometer cavity matching is still required to achieve minimum output poise durations. All the active-passive dye combinations can be used in the hybrid arrangement, with the exception of the Coumarin 6 active dye that cannot be effectively excited by 532-, 511—, or 355-nm radiation. The choice of the saturable absorber in the hybrid technique is less critical than in the purely passive system; therefore, the available wavelength range is broader.
One further advantage of the synchronous-pumping method is that the dyes with very short lifetimes, which would preclude true CW operation, can be made to lase. Synchronously pumped lasers with subpicosecond pulses have been operated up to a wavelength of 1.35μm, and hybrid systems have produced less than 3OO-fs pulses around 97O nm.
The passively mode-locked laser represents the simpliest system in that a mode-locked pump source is not required.
1100 п. зн.
SUPPLEMENTARY READING TASKS
CHOOSING А NONLINEAR CRYSTAL
The key factors for material selection depend not only upon the laser conditions – beam size, beam quality and beam power density – but also upon the crystal's properties, such as phase matching, transparency, damage threshold and temperature stability.
Tunable laser sources ranging from the ultraviolet to the infrared may be achieved by frequency conversion of fixed-wavelength or tunable lasers by using nonlinear crystals. Second harmonic generation (SHG), third harmonic generation (THG) and sum frequency mixing (SFM) have been widely used for frequency up conversion (i.e. the shifting of an optical beam to shorter wavelengths). Optical parametric oscillation (CPO), on the other hand, provides a means for frequency down-conversion with continuously angle or temperature-turned output. Shorter-wavelength (100 to 200 nm) and middle-infrared coherent sources may also be obtained by further Raman-shifting of the nonlinear- crystal-converted output.
Using the direct output of commercial lasers or their harmonics as the pumping sources, ОPО in nonlinear crystals provides a very wide tuning spectrum, from the far infrared to the vacuum ultraviolet.
Materials Selection Rule
The key issues of materials selection for frequency conversion may be summarized as follows:
- high conversion efficiency;
- high damage threshold,
- wide phase-match and transparency range;
- large size with good optical homogeneity;
- low cost and easy fabrication;
- chemically (free of moisture) and mechanically stable.
FEATURES OF THE BBO CRYSTAL
The salient features of the BBO crystal may be readily summarized. First, it has a very wide transparency and phase-matching, angle-tuning range: 190 to 300 nm. Second, it has a high damage threshold – up to 13 GW/cm² at 1.06 m and a 1-ns pulsewidth –and a wide temperature acceptance width of 55°C. This temperature acceptance width is about ten times as large as that of KDP and twice that of KTP. Third, BBO has avery high non-linear coefficient. BBO's d22 value is 4.1 x that of KDP's comparable d36-value, which makes BBO's effective figure of merit 3 to 14 times better that that of KDP for second harmonic generation at 1064 nm.
Fourth, BBO is chemically stable and nearly free of moisture. Fifth, good-quality BBO crystals are commercially available at reasonable prices in sizes as large as l0 x 10 x 10 mm. BBO crystal has been identified as a prime candidate for frequency doubling dye lasers to generate deep-ultraviolet sources. Efficient (relatively speaking, of course), tunable sources in the 201 – to 310-nm range have been reported for phase-matching at room temperature. More recently, а 195-nm source has been achieved using BBO at low temperature for sum frequency mixing the outputs of dye lasers. Commercial dye laser systems with excimer lasers as pumps, using BBO crystals for internal frequency-doubling, are also available. A vacuum-ultraviolet source at 71 nm was recently reported; it involved third harmonic generation, in neon gas, of the BBO-generated 213-nm fifth harmonic of a Nd:YAG laser. Peak output powers of I to 2 W from this fifteenth-harmonic generator have been measured.
Nonlinear crystals play an essential role for the generation of coherent sources in spectral regimes where useful levels of direct output from existing lasers cannot be achieved. An ideal crystal should include all the features of transparency and phase-matching over a wide spectral range; wide angular, temperature and spectral acceptance widths; high damage threshold, high nonlinear coefficient; high degree of homogeneity over relatively large volumes; and chemical and mechanical stability. Finally, crystal cost, in terms of efficiency per dollar, should be reasonable. For high-average-power laser applications, such as laser fusion, developmental goals for such crystals as KDP, LAP and BBO will center on high damage thresholds and large crystal sizes, with diameters up to 20to 40cm.
For low-power laser applications such as diode-pumped lasers and integrated-optics devices, crystals with extremely high nonlinear coefficients (100 to 1,000 times that of KDP) and/or very long aperture length will be required. Along this line, improvements in crystal-growth technology have been emphasized. They include the growth of film and fiber crystals, fabrication of guided-wave devices, growth of new organic crystals and growth of quasi-phase-matching crystals.
CW-PUMPED DYES YIELD ULTRASHORT LASER PULSES
Since its introduction in 1972, the passively mode-locked CW-pumped dye laser has been the source of the shortest pulses with 27 fs being the minimum pulse directly generated from such a system. This 27-fs laser employed the original active/passive dye combination of Rhodamine 6G and 3, 3 , Diethy-loxadicarbocyanine iodide(DODCI). Until 1986, this was the only widely reported passively mode-locked CW-pumped dye-laser scheme and operated in the red region of the spectrum around 620 nm. Since then, many mоrе active/passive dye combinations have been identified, and continuous tunability of femtosecond pulses is now possible throughout most of the visible spectrum.
Absorption and amplification
The mechanism for short-pulse generation in a passively mode-locked dye-laser system relies on the action of saturable absorption and saturable amplification. The saturable absorption attenuates the front or leading edge of the pulse. On encountering the gain medium, the center of the pulse experiences amplification and depletes the available gain such that the rear or trailing edge of the pulse sees no gain and is preferentially suppressed. The ability to generate stable mode-locked pulses is defined by a parameter S, which is given by Rσa/σg, where σg and σa are the dye gain and absorber cross sections, respectively, and R is the ratio of the beam areas in the gain and absorber media.
Reliable mode-locking requires S> 2. In physical terms, this means that the absorption saturates more easily than the gain. As an aid to achieving this condition, the beam area in the gain medium can be larger than that in the absorber through the choice of radius of curvature of the appropriate cavity-folding mirrors.
Argon-ion-laser source
For all the active dyes shown, a CW argon-ion laser can be used as the excitation source. Using Coumarin 6H and Coumarin 102, all-lines UV pumping is required. Improved operation and enchanced output from tnese passively mode-locked systems have been achieved with the introduction of high-UV average-power (up to 5 W) argon-ion-laser systems. In the lasing range550-700 nm the all-lines visible output from a small-frame argon-ion laser producing up to 5 W average pump power is adequate to achieve passively mode-locked operation. Rhodamine 700 and Rhodamine 800 possess principal absorption bands in the region of 650-700 nm; therefore, krypton-ion pumping is preferable.
SUPPLEMENTARY READING TASKS
Taking the measure of light (by Stephen Matthews)
In the early history of the laser, a common method of measuring peak power was to count the number of razor blades through which a pulse could burn. These units were called "Gillettes," and they serve to illustrate how measuring the brightness of light, one of the most intuitive quantities for humans, has long been a problem for technology.
Measuring the instrument
Manufacturers of instruments that measure power and energy have taken pains to make them user-friendly (see Fig. 1). Complex optoelectronic measurements—such as bit-error rate, crosstalk ratio, and so on—can be obtained with a push of a button. But underlying all such complex relationships are fundamental measurements of energy, power, pulse duration, and wavelength. Understanding the physical details of these measurements is particularly essential at the boundaries of current technology—for ultrafast pulses, very high or low light levels, and very short or long wavelengths.
FIGURE 1. Modern instruments for measuring optical signals are designed for ease of use while enabling a variety of sophisticated measurements. Pictured is a representation of a 40-Gbit/s communication signal. Obtaining accurate results, however, still depends on an understanding of the underlying principles of measurement.
The capabilities of an instrument are specified by its resolution, repeatability, and accuracy. Resolution or sensitivity is the minimum quantity that can be discerned in a measurement. The noise present in an instrument during a measurement is a major factor limiting sensitivity. Repeatability quantifies how well an instrument can make one measurement, make a different one, and then return to repeat the first measurement. Accuracy is the ability of an instrument to make a measurement relative to an absolute standard.
As a rule of thumb, the accuracy of an instrument is about ten times greater than its repeatability. Accuracy typically is needed for the most demanding applications. Scheduling routine calibration is particularly important for accurate measurement of optical power and energy, as these instruments in general are more prone to drift over time than other types.
Rookie mistakes
Making a power measurement is often a simple process—just place a photodetector head into a laser beam and read the digital display from the power meter. This simplicity commonly leads to novice mistakes, however. The sensitivity of the detector can easily vary by ±5% or more over its surface. Even miniscule reflections from the detector surface back into the laser will cause power fluctuations.
Another commonly overlooked factor is the effect of transmissive optics on some measurements. And for low light levels or fast pulses, care must be taken to keep connecting cables short to avoid noise pickup or added capacitance, which slows dynamic response. The operation of other instruments, particularly when measuring low signal levels, can also cause spurious results.
Radiometry and photometry
Radiometry is the measurement of optical radiation at wavelengths between 10 m and 1 mm. Photometry is the measurement of light that is detectable by the human eye, and is thus restricted to wavelengths from about 360 to 830 nm. Photometry has an added layer of complexity—measurements are factored by the spectral dependence of human vision. Also, as a practical matter, displays and sources of illumination often have a spatial dependence not found in most nondiode laser sources.
Early measurements of light power compared what the eye perceived to the artificial sources then available. Modern units in the science of photometry are derived from this "standard candle" and are used in the characterization of displays and illumination. The candela is the fundamental SI unit of optical measurement, equivalent in kind to the kilogram and the second.
Most optoelectronic measurements use radiometric units of power and energy familiar from electrical measurements—watts, joules, and so forth. The lumen is the photometric analog of the watt. There are 683 lumens per watt at 555 nm, at which the spectral responsive of the human eye is a maximum (the luminosity curve is set to unity). At other wavelengths, the conversion is scaled to the standard luminosity curve.
The candela can be thought of as the product of lumens and solid angle. There exists a bewildering variety of photometric and radiometric quantities and associated units (Talbots, nits, and blondels, for example). Modern instruments are capable of displaying whatever units are best suited to the application, reducing the burden on the user.
Communication applications such as telecom often use the decibel (dB) to characterize the attenuation of signal transmission. The decibel is a logarithmic ratio of powers: dB = 10 log (Psignal/Pref). The negative sign in attenuation is ignored. When the reference is set to be 1 mW, the signal is then measured in "dBm" units (decibels relative to 1 mW) Decibels are useful units because system losses can be simply added.
The qualities of detectors
Important detector qualities such as spectral response, rise time, and sensitivity differ not only between types but also between different detectors of the same type. These qualities are also influenced by the design of the overall measurement system, including component specification.
Several parameters are used to characterize detector performance. Quantum efficiency—the number of electrons generated per incident photon—is a fundamental parameter that underlies the performance of many types of detectors. Signal-to-noise ratio, which will be discussed in next month's article, is the ultimate figure of merit for a detector.
Thermal detectors
The first measurements of light were made using simple thermometers, and today thermal detectors are still the only practical way to measure some wavelengths and levels of energy. A thermopile consists of a heat-absorbing coated surface connected to a series of thermocouples. The junctions of the thermocouples alternate between a constant temperature reference and the coating (the more thermocouples in series, the greater the sensitivity of the thermopile). The voltage of the thermocouples is calibrated with a known light source incident on the coating.
Given a coating with linear absorption across the spectrum, the thermopile is an all-wavelength detector. Its sensitivity can handle from microwatts of continuous-wave power up to multijoule pulses, with excellent noise performance. The drawback of the thermopile is that it is very slow. The pyroelectric detector, on the other hand, while more expensive and complex, is a fast, broad-spectrum instrument.
The pyroelectric effect refers to the generation of a voltage by heating a ferroelectric crystal. This voltage is capable of producing a current proportional to the change in temperature, and hence to the power incident on the crystal. Since the voltage vanishes when the crystal reaches equilibrium at the new temperature, the signal light must be from a pulsed source, or chopped if the source is continuous.
Unlike semiconductor-based detectors, thermal detectors do not need to be cooled to work in the infrared. Miniature versions of these detectors, such as "microbolorimeters," are being tested in military imaging applications.
Photomultipliers
Photomultiplier tubes (PMTs) were the first detectors to generate electrons directly from photons. The photoemissive element in the tube is the cathode, which is coated with one or more of a variety of alkali compounds. The spectral response of PMTs is determined at longer wavelengths by the photoemissive material and by the transmission of the window in the tube at the blue end.
Electrons emitted by photons striking the cathode are collected by a dynode, which emits two to four electrons for each electron captured. These in turn are captured by the next dynode stage. As many as ten or more stages may be included in a detector. The total amplification can exceed 106, enabling a PMT to detect a single ultraviolet (UV) photon while keeping the noise level very low.
In recent years the PMT has seen increasing competition from the avalanche photodiode (APD), particularly in the visible and infrared range. In response, PMT manufacturers have dramatically reduced the size and relative cost of their tubes. Photomultiplier tubes are now made that fit into a standard TO8 can, and require less than 15 V.
There are literally hundreds of different PMTs, with cathode materials and windows selected to cover portions of the spectrum in the visible and UV. This old technology is still performing in cutting edge applications—for example, PMTs are being used by SETI in its search for alien civilizations.
Photodiodes
The majority of power and energy meters use photodiode detectors. Photodiodes can be operated in either a photovoltaic mode (illuminating the diode produces a voltage) or a photoconductive mode (illuminating the diode produces a current). The photovoltaic mode is simpler, but unfortunately produces a slow and noisy detector.
While increasing the speed of the photodiode, the photoconductive mode may introduce other sources of noise. This illustrates a general difficulty in detector design—there is usually a tradeoff between speed and sensitivity. In the case of the photodiode, integrating the detector with a transimpedance amplifier enables a good balance of both traits.
As would be expected in semiconductor technology, there are highly sophisticated variations of the fundamental p-n junction design. As a simple example, the junction capacitance, which slows detector response, can be reduced by a layer of undoped or "intrinsic material" between the p and n layers, to create a pin photodiode. All but the simplest photodiodes in use today are pin detectors.
Other types of photodiodes
Photodiodes are made from different semiconductor alloys for sensitivity in different spectral regions. Germanium, for example, has a peak response in the near infrared, but it can be doped to produce detectors for wavelengths out to 40 μm. Photodiode detectors for longer wavelengths must be cooled to reduce noise.
The germanium photon-drag detector can handle the high average power of car-bon dioxide (CO2) laser measurements at 10.6 μm. In this design, incident photons transfer their momentum to free carriers in the germanium crystal to produce a current proportional to light power. This detector has low sensitivity but offers a faster response time than some competing pyroelectric instruments.
The material of choice for telecom detectors is indium gallium arsenide (InGaAs), which covers the spectrum from 900 nm to 1.7 μm. While more expensive than germanium devices, these detectors offer lower noise and higher speed. The fastest detectors for use in long-haul telecom applications currently are 60-GHz InGaAs small-area photodiodes packaged with single-mode optical fiber.
The APDs provide high sensitivity and fast response in the infrared, with the result that this detector has become a workhorse in telecom applications, although at a premium price. Its operating principal is the semiconductor equivalent of the PMT—a high bias voltage accelerates a photon-generated electron, which collides with the crystal lattice with enough energy to create additional electrons, which are again accelerated, resulting in a cascade effect and high gain (see Fig. 2).
Дата добавления: 2019-09-13; просмотров: 106; Мы поможем в написании вашей работы! |
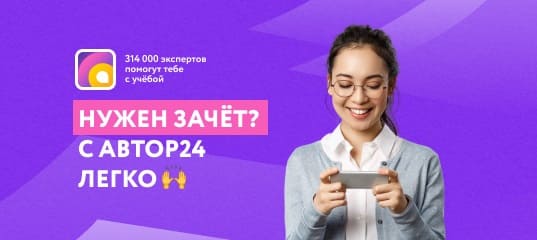
Мы поможем в написании ваших работ!